Bacterial virulence mediated by orthogonal post-translational modification
Many bacterial pathogens secrete virulence factors, also known as effector proteins, directly into host cells. These effectors suppress pro-inflammatory host signaling while promoting bacterial infection. A particularly interesting subset of effectors post-translationally modify host proteins using novel chemistry that is not otherwise found in the mammalian proteome, which we refer to as ‘orthogonal post-translational modification’ (oPTM). In this Review, we profile oPTM chemistry for effectors that catalyze serine/threonine acetylation, phosphate β-elimination, phosphoribosyl-linked ubiquitination, glutamine deamidation, phosphocholination, cysteine methylation, arginine N-acetylglucosaminylation, and glutamine ADP-ribosylation on host proteins. AMPylation, a PTM that could be considered orthogonal until only recently, is also discussed. We further highlight known cellular targets of oPTMs and their resulting biological consequences. Developing a complete understanding of oPTMs and the host cell processes they hijack will illuminate critical steps in the infection process, which can be harnessed for a variety of therapeutic, diagnostic, and synthetic applications.
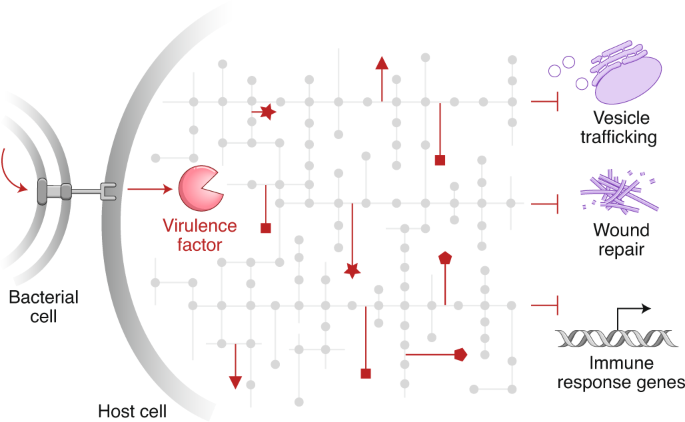
This is a preview of subscription content, access via your institution
Access options
Access Nature and 54 other Nature Portfolio journals
Get Nature+, our best-value online-access subscription
cancel any time
Subscribe to this journal
Receive 12 print issues and online access
265,23 € per year
only 22,10 € per issue
Buy this article
- Purchase on SpringerLink
- Instant access to full article PDF
Prices may be subject to local taxes which are calculated during checkout
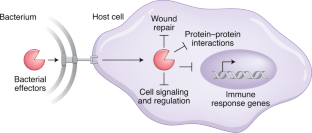
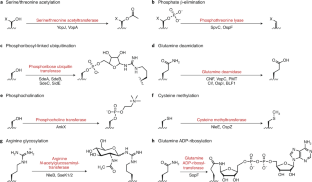
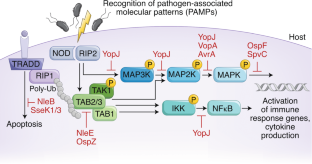
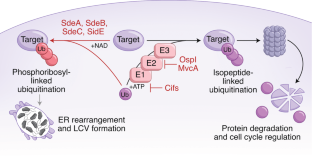
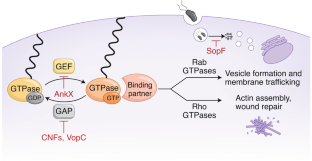
Similar content being viewed by others
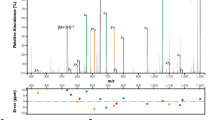
An intra-bacterial activity for a T3SS effector
Article Open access 23 January 2020
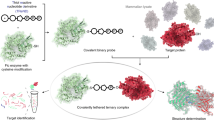
Identification of targets of AMPylating Fic enzymes by co-substrate-mediated covalent capture
Article 06 July 2020
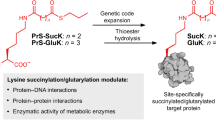
Deciphering functional roles of protein succinylation and glutarylation using genetic code expansion
Article Open access 26 March 2024
References
- Brodsky, I. E. & Medzhitov, R. Targeting of immune signalling networks by bacterial pathogens. Nat. Cell Biol.11, 521–526 (2009). ArticleCASPubMedGoogle Scholar
- Baxt, L. A., Garza-Mayers, A. C. & Goldberg, M. B. Bacterial subversion of host innate immune pathways. Science340, 697–701 (2013). ArticleCASPubMedGoogle Scholar
- Costa, T. R. D. et al. Secretion systems in Gram-negative bacteria: structural and mechanistic insights. Nat. Rev. Microbiol.13, 343–359 (2015). ArticleCASPubMedGoogle Scholar
- Buchrieser, C. et al. The virulence plasmid pWR100 and the repertoire of proteins secreted by the type III secretion apparatus of Shigella flexneri. Mol. Microbiol.38, 760–771 (2000). ArticleCASPubMedGoogle Scholar
- Hubber, A. & Roy, C. R. Modulation of host cell function by Legionella pneumophila type IV effectors. Annu. Rev. Cell Dev. Biol.26, 261–283 (2010). ArticleCASPubMedGoogle Scholar
- Ribet, D. & Cossart, P. Post-translational modifications in host cells during bacterial infection. FEBS Lett.584, 2748–2758 (2010). ArticleCASPubMedGoogle Scholar
- Collier, R. J. & Cole, H. A. Diphtheria toxin subunit active in vitro. Science164, 1179–1181 (1969). ArticleCASPubMedGoogle Scholar
- Mukherjee, S. et al. Yersinia YopJ acetylates and inhibits kinase activation by blocking phosphorylation. Science312, 1211–1214 (2006). This article reports the discovery of Ser/Thr acetylation.ArticleCASPubMedGoogle Scholar
- Mittal, R., Peak-Chew, S. Y. & McMahon, H. T. Acetylation of MEK2 and I kappa B kinase (IKK) activation loop residues by YopJ inhibits signaling. Proc. Natl. Acad. Sci. USA103, 18574–18579 (2006). ArticleCASPubMedPubMed CentralGoogle Scholar
- Ma, K.-W. & Ma, W. YopJ family effectors promote bacterial infection through a unique acetyltransferase activity. Microbiol. Mol. Biol. Rev.80, 1011–1027 (2016). ArticleCASPubMedPubMed CentralGoogle Scholar
- Jones, R. M. et al. Salmonella AvrA coordinates suppression of host immune and apoptotic defenses via JNK pathway blockade. Cell Host Microbe3, 233–244 (2008). ArticleCASPubMedGoogle Scholar
- Trosky, J. E. et al. VopA inhibits ATP binding by acetylating the catalytic loop of MAPK kinases. J. Biol. Chem.282, 34299–34305 (2007). ArticleCASPubMedGoogle Scholar
- Orth, K. et al. Disruption of signaling by Yersinia effector YopJ, a ubiquitin-like protein protease. Science290, 1594–1597 (2000). ArticleCASPubMedGoogle Scholar
- Orth, K. et al. Inhibition of the mitogen-activated protein kinase kinase superfamily by a Yersinia effector. Science285, 1920–1923 (1999). ArticleCASPubMedGoogle Scholar
- Meinzer, U. et al. Yersinia pseudotuberculosis effector YopJ subverts the Nod2/RICK/TAK1 pathway and activates caspase-1 to induce intestinal barrier dysfunction. Cell Host Microbe11, 337–351 (2012). ArticleCASPubMedGoogle Scholar
- Paquette, N. et al. Serine/threonine acetylation of TGFβ-activated kinase (TAK1) by Yersinia pestis YopJ inhibits innate immune signaling. Proc. Natl. Acad. Sci. USA109, 12710–12715 (2012). ArticleCASPubMedPubMed CentralGoogle Scholar
- Mukherjee, S., Hao, Y. H. & Orth, K. A newly discovered post-translational modification—the acetylation of serine and threonine residues. Trends Biochem. Sci.32, 210–216 (2007). ArticleCASPubMedGoogle Scholar
- Zhang, Z. M. et al. Structure of a pathogen effector reveals the enzymatic mechanism of a novel acetyltransferase family. Nat. Struct. Mol. Biol.23, 847–852 (2016). ArticleCASPubMedGoogle Scholar
- Zhang, Z. M. et al. Mechanism of host substrate acetylation by a YopJ family effector. Nat. Plants3, 17115 (2017). ArticleCASPubMedPubMed CentralGoogle Scholar
- Monack, D. M., Mecsas, J., Bouley, D. & Falkow, S. Yersinia-induced apoptosis in vivo aids in the establishment of a systemic infection of mice. J. Exp. Med.188, 2127–2137 (1998). ArticleCASPubMedPubMed CentralGoogle Scholar
- Du, F. & Galán, J. E. Selective inhibition of type III secretion activated signaling by the Salmonella effector AvrA. PLoS Pathog.5, e1000595 (2009). ArticlePubMedPubMed CentralCASGoogle Scholar
- Li, H. et al. The phosphothreonine lyase activity of a bacterial type III effector family. Science315, 1000–1003 (2007). This article reports the discovery of phosphothreonine lyase activity.ArticleCASPubMedGoogle Scholar
- Arbibe, L. et al. An injected bacterial effector targets chromatin access for transcription factor NF-kappaB to alter transcription of host genes involved in immune responses. Nat. Immunol.8, 47–56 (2007). ArticleCASPubMedGoogle Scholar
- Gulig, P. A. et al. Molecular analysis of spv virulence genes of the Salmonella virulence plasmids. Mol. Microbiol.7, 825–830 (1993). ArticleCASPubMedGoogle Scholar
- Zhu, Y. et al. Structural insights into the enzymatic mechanism of the pathogenic MAPK phosphothreonine lyase. Mol. Cell28, 899–913 (2007). ArticleCASPubMedGoogle Scholar
- Chen, L. et al. Structural basis for the catalytic mechanism of phosphothreonine lyase. Nat. Struct. Mol. Biol.15, 101–102 (2008). ArticlePubMedCASGoogle Scholar
- Ke, Z., Smith, G. K., Zhang, Y. & Guo, H. Molecular mechanism for eliminylation, a newly discovered post-translational modification. J. Am. Chem. Soc.133, 11103–11105 (2011). ArticleCASPubMedPubMed CentralGoogle Scholar
- Chambers, K. A., Abularrage, N. S. & Scheck, R. A. Selectivity within a family of bacterial phosphothreonine lyases. Biochemistry57, 3790–3796 (2018). ArticleCASPubMedGoogle Scholar
- Schmutz, C. et al. Systems-level overview of host protein phosphorylation during Shigella flexneri infection revealed by phosphoproteomics. Mol. Cell. Proteomics12, 2952–2968 (2013). ArticleCASPubMedPubMed CentralGoogle Scholar
- Lippmann, J. et al. Bacterial internalization, localization, and effectors shape the epithelial immune response during Shigella flexneri Infection. Infect. Immun.83, 3624–3637 (2015). ArticleCASPubMedPubMed CentralGoogle Scholar
- Chambers, K. A., Abularrage, N. S., Hill, C. J., Khan, I. H. & Scheck, R. A. A chemical probe for dehydrobutyrine. Angew. Chem. Int. Edn Engl.59, 7350–7355 (2020). ArticleCASGoogle Scholar
- Peeler, J. C., Schedin-Weiss, S., Soula, M., Kazmi, M. A. & Sakmar, T. P. Isopeptide and ester bond ubiquitination both regulate degradation of the human dopamine receptor 4. J. Biol. Chem.292, 21623–21630 (2017). ArticleCASPubMedPubMed CentralGoogle Scholar
- Golnik, R., Lehmann, A., Kloetzel, P. M. & Ebstein, F. Major histocompatibility complex (MHC) class I processing of the NY-ESO-1 antigen is regulated by Rpn10 and Rpn13 proteins and immunoproteasomes following non-lysine ubiquitination. J. Biol. Chem.291, 8805–8815 (2016). ArticleCASPubMedPubMed CentralGoogle Scholar
- Bhogaraju, S. et al. Phosphoribosylation of ubiquitin promotes serine ubiquitination and impairs conventional ubiquitination. Cell167, 1636–1649.e13 (2016). This article, along with ref. 35, reports the discovery of phosphoribose ubiquitin transferase activity.ArticleCASPubMedGoogle Scholar
- Kotewicz, K. M. et al. A single Legionella effector catalyzes a multistep ubiquitination pathway to rearrange tubular endoplasmic reticulum for replication. Cell Host Microbe21, 169–181 (2017). This article, along with ref. 34, reports the discovery of phosphoribose ubiquitin transferase activity.ArticleCASPubMedGoogle Scholar
- Qiu, J. et al. Ubiquitination independent of E1 and E2 enzymes by bacterial effectors. Nature533, 120–124 (2016). ArticleCASPubMedPubMed CentralGoogle Scholar
- Dong, Y. et al. Structural basis of ubiquitin modification by the Legionella effector SdeA. Nature557, 674–678 (2018). ArticleCASPubMedGoogle Scholar
- Wang, Y. et al. Structural insights into non-canonical ubiquitination catalyzed by SidE. Cell173, 1231–1243.e16 (2018). ArticleCASPubMedGoogle Scholar
- Akturk, A. et al. Mechanism of phosphoribosyl-ubiquitination mediated by a single Legionella effector. Nature557, 729–733 (2018). ArticleCASPubMedPubMed CentralGoogle Scholar
- Kalayil, S. et al. Insights into catalysis and function of phosphoribosyl-linked serine ubiquitination. Nature557, 734–738 (2018). ArticleCASPubMedPubMed CentralGoogle Scholar
- Qiu, J. et al. A unique deubiquitinase that deconjugates phosphoribosyl-linked protein ubiquitination. Cell Res.27, 865–881 (2017). ArticleCASPubMedPubMed CentralGoogle Scholar
- Wan, M. et al. Deubiquitination of phosphoribosyl-ubiquitin conjugates by phosphodiesterase-domain-containing Legionella effectors. Proc. Natl. Acad. Sci. USA116, 23518–23526 (2019). ArticleCASPubMedPubMed CentralGoogle Scholar
- Shin, D. et al. Regulation of phosphoribosyl-linked serine ubiquitination by deubiquitinases DupA and DupB. Mol. Cell77, 164–179.e6 (2020). ArticleCASPubMedPubMed CentralGoogle Scholar
- Bhogaraju, S. et al. Inhibition of bacterial ubiquitin ligases by SidJ-calmodulin catalysed glutamylation. Nature572, 382–386 (2019). ArticleCASPubMedPubMed CentralGoogle Scholar
- Black, M. H. et al. Bacterial pseudokinase catalyzes protein polyglutamylation to inhibit the SidE-family ubiquitin ligases. Science364, 787–792 (2019). ArticleCASPubMedPubMed CentralGoogle Scholar
- Robinson, N. E. & Robinson, A. B. Use of Merrifield solid phase peptide synthesis in investigations of biological deamidation of peptides and proteins. Biopolymers90, 297–306 (2008). ArticleCASPubMedGoogle Scholar
- Flatau, G. et al. Toxin-induced activation of the G protein p21 Rho by deamidation of glutamine. Nature387, 729–733 (1997). This article, along with ref. 48, reports the discovery of Gln deamidase activity for CNF family effectors.ArticleCASPubMedGoogle Scholar
- Schmidt, G. et al. Gln 63 of Rho is deamidated by Escherichia coli cytotoxic necrotizing factor-1. Nature387, 725–729 (1997). This article, along with ref. 47, reports the discovery of Gln deamidase activity for CNF family effectors.ArticleCASPubMedGoogle Scholar
- Cui, J. et al. Glutamine deamidation and dysfunction of ubiquitin/NEDD8 induced by a bacterial effector family. Science329, 1215–1218 (2010). This article reports the discovery of Gln deamidase activity for Cif family effectors.ArticleCASPubMedPubMed CentralGoogle Scholar
- Marchès, O. et al. Enteropathogenic and enterohaemorrhagic Escherichia coli deliver a novel effector called Cif, which blocks cell cycle G2/M transition. Mol. Microbiol.50, 1553–1567 (2003). ArticlePubMedCASGoogle Scholar
- Taieb, F., Nougayrède, J. P., Watrin, C., Samba-Louaka, A. & Oswald, E. Escherichia coli cyclomodulin Cif induces G2 arrest of the host cell cycle without activation of the DNA-damage checkpoint-signalling pathway. Cell. Microbiol.8, 1910–1921 (2006). ArticleCASPubMedGoogle Scholar
- Samba-Louaka, A. et al. Bacterial cyclomodulin Cif blocks the host cell cycle by stabilizing the cyclin-dependent kinase inhibitors p21 and p27. Cell. Microbiol.10, 2496–2508 (2008). ArticleCASPubMedGoogle Scholar
- Yao, Q. et al. A bacterial type III effector family uses the papain-like hydrolytic activity to arrest the host cell cycle. Proc. Natl. Acad. Sci. USA106, 3716–3721 (2009). ArticleCASPubMedPubMed CentralGoogle Scholar
- Hsu, Y. et al. Structure of the cyclomodulin Cif from pathogenic Escherichia coli. J. Mol. Biol.384, 465–477 (2008). ArticleCASPubMedPubMed CentralGoogle Scholar
- Yu, C. et al. Gln40 deamidation blocks structural reconfiguration and activation of SCF ubiquitin ligase complex by Nedd8. Nat. Commun.6, 10053 (2015). ArticleCASPubMedGoogle Scholar
- Sanada, T. et al. The Shigella flexneri effector OspI deamidates UBC13 to dampen the inflammatory response. Nature483, 623–626 (2012). ArticleCASPubMedGoogle Scholar
- Valleau, D. et al. Discovery of ubiquitin deamidases in the pathogenic arsenal of Legionella pneumophila. Cell Rep.23, 568–583 (2018). ArticleCASPubMedGoogle Scholar
- Gan, N. et al. Legionella pneumophila regulates the activity of UBE2N by deamidase-mediated deubiquitination. EMBO J.39, e102806 (2020). ArticleCASPubMedGoogle Scholar
- Gan, N., Nakayasu, E. S., Hollenbeck, P. J. & Luo, Z. Q. Legionella pneumophila inhibits immune signalling via MavC-mediated transglutaminase-induced ubiquitination of UBE2N. Nat. Microbiol.4, 134–143 (2019). ArticleCASPubMedGoogle Scholar
- Cruz-Migoni, A. et al. A Burkholderia pseudomallei toxin inhibits helicase activity of translation factor eIF4A. Science334, 821–824 (2011). ArticleCASPubMedGoogle Scholar
- Hoffmann, C. et al. The Yersinia pseudotuberculosis cytotoxic necrotizing factor (CNFY) selectively activates RhoA. J. Biol. Chem.279, 16026–16032 (2004). ArticleCASPubMedGoogle Scholar
- Zhang, L. et al. Type III effector VopC mediates invasion for Vibrio species. Cell Rep.1, 453–460 (2012). ArticleCASPubMedPubMed CentralGoogle Scholar
- Mukherjee, S. et al. Modulation of Rab GTPase function by a protein phosphocholine transferase. Nature477, 103–106 (2011). This article reports the discovery of phosphocholine transferase activity.ArticleCASPubMedPubMed CentralGoogle Scholar
- Yarbrough, M. L. et al. AMPylation of Rho GTPases by Vibrio VopS disrupts effector binding and downstream signaling. Science323, 269–272 (2009). ArticleCASPubMedGoogle Scholar
- Worby, C. A. et al. The fic domain: regulation of cell signaling by adenylylation. Mol. Cell34, 93–103 (2009). ArticleCASPubMedPubMed CentralGoogle Scholar
- Campanacci, V., Mukherjee, S., Roy, C. R. & Cherfils, J. Structure of the Legionella effector AnkX reveals the mechanism of phosphocholine transfer by the FIC domain. EMBO J.32, 1469–1477 (2013). ArticleCASPubMedPubMed CentralGoogle Scholar
- Engel, P. et al. Adenylylation control by intra- or intermolecular active-site obstruction in Fic proteins. Nature482, 107–110 (2012). ArticleCASPubMedGoogle Scholar
- Sreelatha, A. et al. Protein AMPylation by an evolutionarily conserved pseudokinase. Cell175, 809–821.e819 (2018). ArticleCASPubMedPubMed CentralGoogle Scholar
- Gavriljuk, K. et al. Unraveling the phosphocholination mechanism of the Legionella pneumophila enzyme AnkX. Biochemistry55, 4375–4385 (2016). ArticleCASPubMedGoogle Scholar
- Goody, P. R. et al. Reversible phosphocholination of Rab proteins by Legionella pneumophila effector proteins. EMBO J.31, 1774–1784 (2012). ArticleCASPubMedPubMed CentralGoogle Scholar
- Yao, Q. et al. Structure and specificity of the bacterial cysteine methyltransferase effector NleE suggests a novel substrate in human DNA repair pathway. PLoS Pathog.10, e1004522 (2014). ArticlePubMedPubMed CentralCASGoogle Scholar
- Zhang, L. et al. Cysteine methylation disrupts ubiquitin-chain sensing in NF-κB activation. Nature481, 204–208 (2011). This article reports the discovery of cysteine methylation.ArticlePubMedCASGoogle Scholar
- Zhang, Y., Mühlen, S., Oates, C. V., Pearson, J. S. & Hartland, E. L. Identification of a distinct substrate-binding domain in the bacterial cysteine methyltransferase effectors NleE and OspZ. J. Biol. Chem.291, 20149–20162 (2016). ArticleCASPubMedPubMed CentralGoogle Scholar
- Ding, J. et al. Structural and functional insights into host death domains inactivation by the bacterial arginine GlcNAcyltransferase effector. Mol Cell74, 922–935.e926 (2019). ArticleCASPubMedGoogle Scholar
- Li, S. et al. Pathogen blocks host death receptor signalling by arginine GlcNAcylation of death domains. Nature501, 242–246 (2013). This article, along with ref. 76, reports the discovery of arginine GlcNAcylation.ArticleCASPubMedGoogle Scholar
- Pearson, J. S. et al. A type III effector antagonizes death receptor signalling during bacterial gut infection. Nature501, 247–251 (2013). This article, along with ref. 75, reports the discovery of arginine GlcNAcylation.ArticleCASPubMedPubMed CentralGoogle Scholar
- Newson, J. P. M. et al. Salmonella effectors SseK1 and SseK3 target death domain proteins in the TNF and TRAIL signaling pathways. Mol. Cell. Proteomics18, 1138–1156 (2019). ArticleCASPubMedPubMed CentralGoogle Scholar
- Xu, Y. et al. A bacterial effector reveals the V-ATPase-ATG16L1 axis that initiates xenophagy. Cell178, 552–566.e520 (2019). This article reports the discovery of glutamine ADP-ribosylation.ArticleCASPubMedGoogle Scholar
- Lim, D. V., Simpson, J. M., Kearns, E. A. & Kramer, M. F. Current and developing technologies for monitoring agents of bioterrorism and biowarfare. Clin. Microbiol. Rev.18, 583–607 (2005). ArticleCASPubMedPubMed CentralGoogle Scholar
- Enninga, J., Mounier, J., Sansonetti, P. & Tran Van Nhieu, G. Secretion of type III effectors into host cells in real time. Nat. Methods2, 959–965 (2005). ArticleCASPubMedGoogle Scholar
- Isberg, R. R., O’Connor, T. J. & Heidtman, M. The Legionella pneumophila replication vacuole: making a cosy niche inside host cells. Nat. Rev. Microbiol.7, 13–24 (2009). ArticleCASPubMedGoogle Scholar
- Mitchell, A., Wei, P. & Lim, W. A. Oscillatory stress stimulation uncovers an Achilles’ heel of the yeast MAPK signaling network. Science350, 1379–1383 (2015). ArticleCASPubMedPubMed CentralGoogle Scholar
- Zhao, J. et al. Bioorthogonal engineering of bacterial effectors for spatial-temporal modulation of cell signaling. ACS Cent. Sci.5, 145–152 (2019). ArticleCASPubMedGoogle Scholar
- Wei, P. et al. Bacterial virulence proteins as tools to rewire kinase pathways in yeast and immune cells. Nature488, 384–388 (2012). ArticleCASPubMedPubMed CentralGoogle Scholar
- Ochtrop, P., Ernst, S., Itzen, A. & Hedberg, C. Exploring the substrate scope of the bacterial phosphocholine transferase AnkX for versatile protein functionalization. ChemBioChem20, 2336–2340 (2019). ArticleCASPubMedGoogle Scholar
- Heller, K. et al. Covalent protein labeling by enzymatic phosphocholination. Angew. Chem. Int. Edn Engl.54, 10327–10330 (2015). ArticleCASGoogle Scholar
- Baker, S. J., Payne, D. J., Rappuoli, R. & De Gregorio, E. Technologies to address antimicrobial resistance. Proc. Natl. Acad. Sci. USA115, 12887–12895 (2018). ArticleCASPubMedPubMed CentralGoogle Scholar
- Allen, R. C., Popat, R., Diggle, S. P. & Brown, S. P. Targeting virulence: can we make evolution-proof drugs? Nat. Rev. Microbiol.12, 300–308 (2014). ArticleCASPubMedGoogle Scholar
- Dickey, S. W., Cheung, G. Y. C. & Otto, M. Different drugs for bad bugs: antivirulence strategies in the age of antibiotic resistance. Nat. Rev. Drug Discov.16, 457–471 (2017). ArticleCASPubMedGoogle Scholar
- Lakemeyer, M., Zhao, W., Mandl, F. A., Hammann, P. & Sieber, S. A. Thinking outside the box-novel antibacterials to tackle the resistance crisis. Angew. Chem. Int. Edn Engl.57, 14440–14475 (2018). ArticleCASGoogle Scholar
- McShan, A. C. & De Guzman, R. N. The bacterial type III secretion system as a target for developing new antibiotics. Chem. Biol. Drug Des.85, 30–42 (2015). ArticleCASPubMedPubMed CentralGoogle Scholar
- Kauppi, A. M., Nordfelth, R., Uvell, H., Wolf-Watz, H. & Elofsson, M. Targeting bacterial virulence: inhibitors of type III secretion in Yersinia. Chem. Biol.10, 241–249 (2003). ArticleCASPubMedGoogle Scholar
- Slepenkin, A., Chu, H., Elofsson, M., Keyser, P. & Peterson, E. M. Protection of mice from a Chlamydia trachomatis vaginal infection using a Salicylidene acylhydrazide, a potential microbicide. J. Infect. Dis.204, 1313–1320 (2011). ArticleCASPubMedPubMed CentralGoogle Scholar
- Lewallen, D. M. et al. Inhibiting AMPylation: a novel screen to identify the first small molecule inhibitors of protein AMPylation. ACS Chem. Biol.9, 433–442 (2014). ArticleCASPubMedGoogle Scholar
- Salomon, D. & Orth, K. What pathogens have taught us about posttranslational modifications. Cell Host Microbe14, 269–279 (2013). ArticleCASPubMedPubMed CentralGoogle Scholar
- Kingdon, H. S., Shapiro, B. M. & Stadtman, E. R. Regulation of glutamine synthetase. 8. ATP: glutamine synthetase adenylyltransferase, an enzyme that catalyzes alterations in the regulatory properties of glutamine synthetase. Proc. Natl. Acad. Sci. USA58, 1703–1710 (1967). ArticleCASPubMedPubMed CentralGoogle Scholar
- Müller, M. P. et al. The Legionella effector protein DrrA AMPylates the membrane traffic regulator Rab1b. Science329, 946–949 (2010). ArticlePubMedGoogle Scholar
- Ham, H. et al. Unfolded protein response-regulated Drosophila Fic (dFic) protein reversibly AMPylates BiP chaperone during endoplasmic reticulum homeostasis. J. Biol. Chem.289, 36059–36069 (2014). ArticleCASPubMedPubMed CentralGoogle Scholar
- Sanyal, A. et al. A novel link between Fic (filamentation induced by cAMP)-mediated adenylylation/AMPylation and the unfolded protein response. J. Biol. Chem.290, 8482–8499 (2015). ArticleCASPubMedPubMed CentralGoogle Scholar
- Broncel, M., Serwa, R. A., Bunney, T. D., Katan, M. & Tate, E. W. Global profiling of Huntingtin-associated protein E (HYPE)-mediated AMPylation through a chemical proteomic approach. Mol. Cell. Proteomics15, 715–725 (2016). ArticleCASPubMedGoogle Scholar
- Grammel, M., Luong, P., Orth, K. & Hang, H. C. A chemical reporter for protein AMPylation. J. Am. Chem. Soc.133, 17103–17105 (2011). ArticleCASPubMedPubMed CentralGoogle Scholar
- Kielkowski, P. et al. FICD activity and AMPylation remodelling modulate human neurogenesis. Nat. Commun.11, 517 (2020). ArticleCASPubMedPubMed CentralGoogle Scholar
Acknowledgements
This work was supported in part by a Tufts Collaborates Award to R.A.S. The authors gratefully acknowledge K. Allen, D. Walt, and J. Kritzer for helpful feedback regarding the preparation of this manuscript.